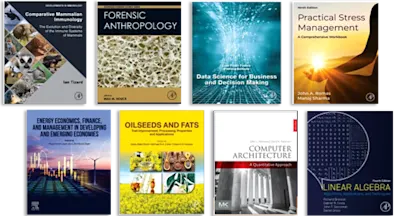
Comprehensive Biomaterials
- 1st Edition - August 24, 2011
- Imprint: Elsevier Science
- Editors: Paul Ducheyne, Paul Ducheyne, Kevin Healy, Dietmar W. Hutmacher, David W. Grainger, C. James Kirkpatrick
- Language: English
- eBook ISBN:9 7 8 - 0 - 0 8 - 0 9 1 5 1 4 - 2
- eBook ISBN:9 7 8 - 0 - 0 8 - 0 5 5 2 9 4 - 1
Comprehensive Biomaterials brings together the myriad facets of biomaterials into one, major series of six edited volumes that would cover the field of biomaterials in a major, ex… Read more
Purchase options
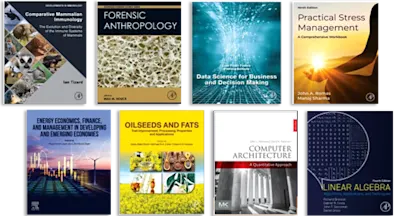
Comprehensive Biomaterials brings together the myriad facets of biomaterials into one, major series of six edited volumes that would cover the field of biomaterials in a major, extensive fashion:
Volume 1: Metallic, Ceramic and Polymeric BiomaterialsVolume 2: Biologically Inspired and Biomolecular MaterialsVolume 3: Methods of AnalysisVolume 4: Biocompatibility, Surface Engineering, and Delivery Of Drugs, Genes and Other MoleculesVolume 5: Tissue and Organ EngineeringVolume 6: Biomaterials and Clinical Use
Experts from around the world in hundreds of related biomaterials areas have contributed to this publication, resulting in a continuum of rich information appropriate for many audiences. The work addresses the current status of nearly all biomaterials in the field, their strengths and weaknesses, their future prospects, appropriate analytical methods and testing, device applications and performance, emerging candidate materials as competitors and disruptive technologies, and strategic insights for those entering and operational in diverse biomaterials applications, research and development, regulatory management, and commercial aspects. From the outset, the goal was to review materials in the context of medical devices and tissue properties, biocompatibility and surface analysis, tissue engineering and controlled release. It was also the intent both, to focus on material properties from the perspectives of therapeutic and diagnostic use, and to address questions relevant to state-of-the-art research endeavors.
- Reviews the current status of nearly all biomaterials in the field by analyzing their strengths and weaknesses, performance as well as future prospects
- Presents appropriate analytical methods and testing procedures in addition to potential device applications
- Provides strategic insights for those working on diverse application areas such as R&D, regulatory management, and commercial development
This work is of interest to any student, researcher or engineer working in biomaterials, medicinal research, cell biology, tissue engineering, tissue physiology, regenerative medicine, microfabrication, and biomedical devices and applications
Editor-in-Chief
Co-Editors
Editor-in-Chief Biography
Co-Editor Biographies
Preface
Foreword
Permission Acknowledgments
1.101. Biomaterials
1.102. Metals for Use in Medicine
Abbreviations
1.102.1. Introduction
1.102.2. General Requirements for Long-Term Implantation
1.102.3. Key Metallurgy Concepts
1.102.4. Chemical Composition and Structure
1.102.5. Mechanical Properties
1.102.6. Processing Effects
1.102.7. Future Developments
1.102.8. Summary
1.103. Electrochemical Behavior of Metals in the Biological Milieu
Abbreviations
Acknowledgments
1.103.1. Introduction
1.103.2. Metals Currently Used in Medical Devices
1.103.3. Metallic Biocompatibility
1.103.4. The Biological Milieu
1.103.5. Basic Electrochemistry Concepts
1.103.6. Passive Oxide Films and Semiconducting Electrochemistry
1.103.7. Electrical Double Layer
1.103.8. Electrochemical Impedance Spectroscopy (EIS) of Metallic Biomaterials
1.103.9. Mechanically Assisted Corrosion
1.103.10. Effects of Prior Electrochemical History
1.103.11. Oxide Film Structure and Formation
1.103.12. Effects of Solution Redox System
1.103.13. Biological Consequences: Oxidation and Reduction
1.103.14. Summary
1.103.15. Appendix: Derivation of Mott–Schottky Equation
1.104. Shape Memory Alloys for Use in Medicine
Abbreviations
1.104.1. Introduction
1.104.2. Fundamentals of Shape Memory Systems
1.104.3. Practical SMAs
1.104.4. Manufacturing, Processing, and Performance of Nitinol
1.104.5. Minimally Invasive Device Applications for Nitinol
1.104.6. Orthodontic Applications for Nitinol
1.104.7. Orthopedic Applications for Nitinol
1.104.8. Clinical Imaging of Nitinol Medical Devices
1.104.9. Long-Term Durability and Biocompatibility of Nitinol
1.104.10. Summary and Future Directions
1.105. Alumina
Abbreviations
1.105.1. Introduction
1.105.2. Properties of Alumina
1.105.3. Clinical Application of Alumina Ceramics
1.106. Zirconia as a Biomaterial
Abbreviations
1.106.1. Introduction
1.106.2. Crystallography and Phase Transformation of Zirconia
1.106.3. Different Types of Zirconia and Zirconia-Based Composites
1.106.4. The Use of Zirconia as a Biomaterial: Current State of the Art
1.106.5. Future Directions
1.106.6. Conclusion
1.106.7. Further Reading
1.107. Carbon and Diamond
Abbreviations
1.107.1. Introduction
1.107.2. Pyrolytic Carbon
1.107.3. Diamond-Like Carbon
1.107.4. Microcrystalline, Nanocrystalline, and Ultrananocrystalline Diamond
1.107.5. Summary of Carbon and Diamond
1.108. Wear-Resistant Ceramic Films and Coatings
Abbreviations
1.108.1. Introduction
1.108.2. State of the Art – Processing, Microstructure, Biocompatibility, and Mechanical Properties of Ceramic Coatings
1.108.3. Future Trends
1.109. Bioactive Ceramics
Abbreviations
1.109.1. Bioactivity
1.109.2. Bone Formation at the Interface with Bioactive Materials
1.109.3. Testing Bioactivity In Vitro
1.109.4. Methods of Analysis of the Dissolution–Precipitation Reaction
1.109.5. Bioactive Glasses
1.109.6. Bioactive Glass Ceramics
1.109.7. Calcium Phosphate Ceramics
1.109.8. Silica-Calcium Phosphate Nanocomposite
1.109.9. Silica-Xerogel
1.109.10. Conclusion
1.110. Bioactive Glass-Ceramics
Abbreviations
1.110.1. AW Glass-Ceramic
1.110.2. Apatite–Mica Glass-Ceramics
1.110.3. Apatite–Mullite Glass-Ceramics
1.112. Calcium Phosphate Coatings
1.112.1. Introduction
1.112.2. Calcium Phosphates
1.112.3. Titanium Implants
1.112.4. Plasma Spraying of CaP Coatings
1.112.5. Electrochemical Deposition of CaP Coatings
1.112.6. Biomimetic CaP Coatings
1.112.7. Conclusion and Perspectives
1.113. Bioactive Layer Formation on Metals and Polymers
Abbreviations
1.113.1. Introduction
1.113.2. Bioactive Layers
1.113.3. Future Perspectives
1.113.4. Additional Reading
1.114. Bioactivity: Mechanisms
Abbreviations
Acknowledgments
1.114.1. Introduction
1.114.2. Mechanisms of Bioactive Behavior
1.114.3. Mechanisms of Biodegradation of Bioactive Ceramics
1.114.4. Summary
1.115. Calcium Phosphates for Cell Transfection
Abbreviations
1.115.1. Introduction
1.115.2. Applications of Bioceramic Cell Transfection
1.115.3. The Role of Bioceramic Characteristics for their Applications
1.115.4. Traditional Use of Calcium Phosphate for Cell Transfection
1.115.5. Hydroxylapatite Ceramics for Cell Transfection
1.115.6. Perspectives and Material Evolution
1.116. Bioactive Ceramics: Cements
Abbreviations
1.116.1. Introduction
1.116.2. Calcium Sulfate
1.116.3. Portland Cement and MTA
1.116.4. Apatite Cement
1.116.5. Brushite Cement
1.116.6. Future Direction
1.117. Phosphate-Based Glasses
Abbreviations
1.117.1. Phosphate Glasses: Physical Properties and Processing
1.117.2. Biological Properties of Phosphate Glasses
1.117.3. Therapeutic Actions of Phosphate Glasses
1.118. Calcium Phosphate Ceramics with Inorganic Additives
Abbreviations
1.118.1. Introduction
1.118.2. Bone
1.118.3. Current Methods in Bone Regeneration
1.118.4. Improvement of Synthetic Bone Graft Substitutes
1.118.5. Trace Elements in Bone Metabolism and Processes Related to Bone Formation
1.118.6. Inorganic Additives in Calcium Phosphate Ceramics
1.118.7. Future Perspectives
1.119. Silicon-Containing Apatites
Abbreviations
1.119.1. Introduction
1.119.2. Synthesis and Characterization of Silicon-Containing Apatites
1.119.3. In Vitro and In Vivo Studies
1.119.4. Mechanisms of Enhanced Biological Response
1.119.5. Other Silicon-Containing Calcium Phosphate Ceramics
1.119.6. Conclusions and Future Directions
1.120. Synthetic Bone Grafts: Clinical Use
Abbreviations
1.120.1. Introduction
1.120.2. A Brief History of Bone Grafts
1.120.3. Synthetic Bone Graft Substitutes
1.120.4. Conclusion
1.121. Polymer Fundamentals: Polymer Synthesis
1.121.1. Introduction to Polymer Science
1.121.2. Polycondensation
1.121.3. Addition Polymerization
1.121.4. Polymer Reactions
1.121.5. Conclusion
1.122. Structural Biomedical Polymers (Nondegradable)
1.122.1. Historical Overview
1.122.2. Classifications of Medical Polymers and Their Applications
1.122.3. Designing Structural Implants with Polymers
1.122.4. Summary
1.123. Degradable Polymers
Abbreviations
1.123.1. Introduction
1.123.2. Overview of PLGA Copolymers
1.123.3. Parameters Affecting Degradation Rate of PLGA
1.123.4. Biocompatibility of PLGA
1.123.5. Applications of PLGA Across Length Scales
1.123.6. Rationale for Development of PPEs
1.123.7. Structure–Function Relationships of PPEs
1.123.8. Toxicity and Biocompatibility of PPEs
1.123.9. Design of PPE Drug Carriers
1.123.10. Design of Thermoresponsive PPEs
1.123.11. Design of PPE and PPA Gene Carriers
1.123.12. Conclusion
1.124. Polymer Films Using LbL Self-Assembly
Abbreviations
1.124.1. Introduction: Strategies for Surface Modification with Polymers
1.124.2. Fundamentals of Multilayer Formation: LbL Thin Films
1.124.3. Conclusions
1.126. Shape-Memory Polymers
Abbreviations
1.126.1. Fundamental Principles of Shape-Memory Polymers and of the Quantification of Shape-Memory Properties
1.126.2. Synthesis and Properties of Selected Examples of SMP Intended for Biomedical Applications
1.126.3. Multifunctional SMPs by Integrating Hydrolytic Degradability or Controlled Drug Release Capability
1.126.4. Biomedical Applications of SMPs
1.127. Electrospinning and Polymer Nanofibers: Process Fundamentals
Abbreviations
1.127.1. Introduction
1.127.2. Process Description
1.127.3. Mechanics: Electrostatics and Hydrodynamics
1.127.4. Products of Electrospinning
1.127.5. Summary and Conclusion
1.128. Fluorinated Biomaterials
Abbreviations
Acknowledgments
1.128.1. Introduction
1.128.2. Fluorinated Polymer Chemical and Physical Properties
1.128.3. Fluoropolymers
1.128.4. Biomedical Applications of Fluorinated Biomaterials
1.128.5. Conclusion and Perspectives
1.129. Engineering the Biophysical Properties of Basement Membranes into Biomaterials: Fabrication and Effects on Cell Behavior
Abbreviations
Acknowledgments
1.129.1. Introduction
1.129.2. Basement Membrane
1.129.3. Nanostructure Fabrication
1.129.4. Cellular Response to Topographic Cues with Dimensions from Nano- to Micron-Scales
1.130. Electroactive Polymeric Biomaterials
Abbreviations
Acknowledgments
1.130.1. Introduction
1.130.2. Conjugated Polymer Electrode Coatings
1.130.3. Conjugated Polymers on Devices
1.130.4. Implantable Modification of Conjugated Polymers
1.130.5. Conjugated Polymer-Based Drug Delivery
1.130.6. Synthesis of Conducting Polymers In Vivo
1.130.7. Summary and Future Outlook
1.131. Superporous Hydrogels for Drug Delivery Systems
Abbreviations
1.131.1. Introduction
1.131.2. Hydrogels in Drug Delivery
1.131.3. Superporous Hydrogels
1.131.4. SPH Synthesis
1.131.5. SPH Properties
1.131.6. SPH Generations
1.131.7. SPH Scale Up
1.131.8. SPH Stability
1.131.9. SPH Safety
1.131.10. SPH Platform Design for Drug Delivery
1.131.11. SPH in Drug Delivery and Other Areas
1.131.12. Conclusions
1.132. Dynamic Hydrogels
Abbreviations
1.132.1. Introduction
1.132.2. Functional Modes/General Dynamic Mechanisms
1.132.3. Specific Stimuli and Response Mechanisms
1.132.4. Biomedical Applications
1.132.5. Future Directions
2.201. Bio-inspired Silica Nanomaterials for Biomedical Applications
Abbreviations
Acknowledgment
2.201.1. Introduction
2.201.2. Biosilicification
2.201.3. Bio-inspired Silica Synthesis for Biomedical Applications
2.201.4. Perspectives and Challenges
2.201.5. Conclusion
2.202. Engineering Viruses For Gene Therapy
Abbreviations
2.202.1. Introduction
2.202.2. Viral Engineering
2.202.3. Design of Artificial Viruses
2.202.4. Conclusions and Future Work
2.203. Protein-Engineered Biomaterials: Synthesis and Characterization
Abbreviations
2.203.1. Introduction
2.203.2. Rational Design and Modular Building Blocks
2.203.3. Synthesis and Purification
2.203.4. Postsynthesis Materials Processing
2.203.5. Characterization
2.203.6. Biological Interactions and Immunogenicity
2.203.7. Future Directions and Conclusions
2.204. Peptoids: Synthesis, Characterization, and Nanostructures
Abbreviations
2.204.1. Introduction
2.204.2. Synthesis
2.204.3. Peptoid Structure and Characterization
2.204.4. Combinatorial Discovery of Peptoid Ligands
2.204.5. Drug Discovery
2.204.6. Cellular Delivery/Uptake Vectors
2.204.7. Biomimetic Materials
2.204.8. Summary and Future Directions
2.205. Self-Assembling Biomaterials
Abbreviations
2.205.1. Introduction
2.205.2. Planar Self-Assembling Systems
2.205.3. 3D Self-Assembling Systems
2.205.4. Modulating the Mechanics of Self-Assembling Systems
2.205.5. Advantages Provided by Self-Assembled Systems for Biomaterials Applications
2.205.6. Immune and Inflammatory Responses to Self-Assembling Materials
2.205.7. In vivo Applications of Self-Assembled Biomaterials
2.205.8. Concluding Remarks
2.206. Phages as Tools for Functional Nanomaterials Development
Abbreviations
2.206.1. Introduction
2.206.2. Phages for Inorganic–Organic Hybrid Materials
2.206.3. Phage for Energy Materials
2.206.4. Phage for Sensing Materials
2.206.5. Phage for Biomedical Application
2.206.6. Summary and Future Perspectives
2.207. Extracellular Matrix: Inspired Biomaterials
Abbreviations
2.207.1. Introduction
2.207.2. Overview of ECM Structure and Function
2.207.3. Types of ECM Mimicry
2.207.4. Future Directions
2.208. Artificial Extracellular Matrices to Functionalize Biomaterial Surfaces
Abbreviations
2.208.1. Introduction
2.208.2. Components to Be Used for aECM
2.208.3. Biological Interaction Profiles of aECM and Their Components
2.208.4. Preparation and Structure of aECM
2.208.5. Biochemical Characterization of aECM
2.208.6. Immobilization of aECM
2.208.7. Cell Biological Effects of aECM
2.208.8. Results from Animal Experiments
2.208.9. Conclusions and Outlook
2.209. Materials as Artificial Stem Cell Microenvironments
Abbreviations
2.209.1. Introduction
2.209.2. The Adult Stem Cell and Its Niche
2.209.3. Naturally Derived ECM Components for In Vitro Stem Cell Manipulation
2.209.4. Engineered Substrates as Artificial Stem Cell Niches
2.209.5. Topographically Patterned Substrates as Versatile Stem Cell Microenvironments
2.209.6. Biomaterials Approaches to Emulate Stem Cell Niches in 3D
2.209.7. Conclusions
2.210. Bone as a Material
Abbreviations
2.210.1. Introduction
2.210.2. Bone Composition
2.210.3. Bone Formation
2.210.4. Bone Structure and Hierarchical Organization
2.210.5. Bone Mechanical Behavior
2.210.6. Bone as a Dynamic Adaptive Material
2.210.7. Bone as a Material During Disease and Drug Treatment
2.210.8. Conclusion
2.211. Polymers of Biological Origin
Abbreviations
2.211.1. Introduction
2.211.2. Natural-Based Polymeric Systems
2.211.3. Processing of TE Scaffolds
2.211.4. Cell Encapsulation in Injectable Biodegradable Hydrogels for TE Applications
2.211.5. Final Remarks
2.212. Silk Biomaterials
Acknowledgments
2.212.1. Overview
2.212.2. Silk Film Biomaterials
2.212.3. Silk Sponge Scaffold Biomaterials
2.212.4. Silk Nanofiber Biomaterials
2.212.5. Silk Hydrogel Biomaterials
2.212.6. Silk Microsphere and Nanoparticle Biomaterials
2.212.7. Silk Optical Biomaterials
2.212.8. Other Silk Materials
2.212.9. Conclusions
2.213. Chitosan
Abbreviations
2.213.1. Sources, Analysis, and Properties
2.213.2. Processing
2.213.3. Biomedical Applications
2.213.4. Future Prospects
2.214. Hyaluronic Acid
Abbreviations
Acknowledgments
2.214.1. Introduction
2.214.2. Production of HA
2.214.3. Analysis and Industry Standards
2.214.4. Chemical Modification of HA
2.214.5. Medical Applications of HA
2.214.6. Future Perspectives and Conclusions
2.215. Collagen: Materials Analysis and Implant Uses
Abbreviations
Acknowledgments
2.215.1. Origins of Collagen and Role in Animal Physiology
2.215.2. The Biochemical Fingerprint of Collagen
2.215.3. Sources of Collagen
2.215.4. Collagen: Purification and Analysis
2.215.5. Biomedical Applications of Collagen
2.215.6. Outlook and Future for Collagen Materials
2.216. Collagen–GAG Materials
Abbreviations
2.216.1. Introduction
2.216.2. Fabrication of Collagen–GAG Materials
2.216.3. Characterization of Collagen–GAG Materials
2.216.4. In Vitro Applications
2.216.5. In Vivo Applications
2.216.6. Conclusions
2.217. Fibrin
Abbreviations
2.217.1. Historical Perspective
2.217.2. Composition, Structure, and Properties
2.217.3. Fibrin Use as a Delivery System
2.217.4. Fibrin in Tissue Engineering Applications
2.217.5. Fibrin in Clinical Practice
2.217.6. Conclusion
2.218. Elastin Biopolymers
Abbreviations
2.218.1. Recombinant Human Tropoelastin-Based Constructs
2.218.2. The Elastin-Like Recombinamers
2.218.3. Animal-Derived Solubilized Elastin
2.219. Biophysical Analysis of Amyloid Formation
Abbreviations
Acknowledgments
2.219.1. Introduction
2.219.2. Monomer Folding
2.219.3. Aggregation Intermediates
2.219.4. Mature Amyloid Fibrils
2.219.5. Conclusion
2.220. Extracellular Matrix as Biomimetic Biomaterial: Biological Matrices for Tissue Regeneration
Abbreviations
2.220.1. Extracellular Matrix
2.220.2. Materials Applied in TE
2.220.3. Induction of Graft Vascularization
2.220.4. Biological Vascularized Scaffold
2.220.5. Summary and Future Directions
2.221. Decellularized Scaffolds
Abbreviations
2.221.1. Introduction
2.221.2. Decellularization Methodology
2.221.3. Origin of Decellularized Scaffolds and their Applications
2.221.4. Concluding Remarks
2.223. Bacterial Cellulose as Biomaterial
Abbreviations
2.223.1. Introduction
2.223.2. Bacterial Cellulose: Growth
2.223.3. Modification of Nanostructure
2.223.4. Engineering of Morphology
2.223.5. Shaping in 3D Structure
2.223.6. Biocompatibility
2.223.7. Biomechanics
2.223.8. Cell Interactions and Migration
2.223.9. Biomedical Applications
2.223.10. Future Outlook
3.301. Surface Analysis and Biointerfaces: Vacuum and Ambient In Situ Techniques
Abbreviations
Acknowledgments
3.301.1. Introduction
3.301.2. Ambient Analytical Methods
3.301.3. Ultrahigh Vacuum Surface Analytical Techniques
3.301.4. Next Challenges: Nanomaterial Surfaces
3.301.5. Conclusions
3.302. Atomic Force Microscopy
Abbreviations
3.302.1. Introduction
3.302.2. Fundamentals of the AFM
3.302.3. Force–Distance Measurement and Force Mapping
3.302.4. Nanoindentation and Measurement of Near-Surface Nanomechanical Properties of Polymeric Biomaterials
3.302.5. Probing Molecular Interaction and Recognition Sites on Biosurfaces
3.302.6. Limitations and Perspectives
3.303. Proteomic and Advanced Biochemical Techniques to Study Protein Adsorption
Abbreviations
3.303.1. Introduction
3.303.2. Unbiased Analysis of Adsorbed Proteins by Polyacrylamide Gel Electrophoresis
3.303.3. Analysis of Adsorbed Proteins by 2DPAGE
3.303.4. Quantification of Protein Amounts by Mass Spectrometry
3.303.5. Proteomics to Study Protein Denaturation on Surfaces
3.303.6. Challenges of the Proteomics Approach
3.303.7. Conclusions
3.304. Developments in High-Resolution CT: Studying Bioregeneration by Hard X-ray Synchrotron-Based Microtomography
Abbreviations
Acknowledgments
3.304.1. Hard X-ray Microimaging
3.304.2. Comparative Animal Study of Bioceramic-Supported Bone Regeneration
3.304.3. Bone Regeneration After Sinus Floor Augmentation in Humans
3.304.4. Summary
3.305. Biomedical Thin Films: Mechanical Properties
Abbreviations
Acknowledgments
3.305.1. Introduction
3.305.2. Coating Techniques
3.305.3. Thin Film Mechanical Testing: Methods
3.305.4. Concluding Remarks
3.306. Microindentation
Abbreviations
3.306.1. Introduction
3.306.2. Overview of Nanoindentation Technique
3.306.3. Application of Nanoindentation to Biomaterials
3.306.4. Summary
3.307. Finite Element Analysis in Bone Research: A Computational Method Relating Structure to Mechanical Function
Abbreviations
3.307.1. Introduction
3.307.2. Macroscale: Whole Bone
3.307.3. Mesoscale: Trabecular Bone
3.307.4. Microscale: Ultrastructural Features
3.307.5. Nanoscale: Mineral and Collagen
3.307.6. Conclusions and Future Directions
3.308. The Mechanics of Native and Engineered Cardiac Soft Tissues
Abbreviations
Acknowledgment
3.308.1. Introduction
3.308.2. Heart Valve Tissues
3.308.3. Myocardium
3.308.4. Constitutive Models of Soft Tissues
3.308.5. Emulating Native Tissue Mechanical Behavior in Engineered Tissues
3.309. Fluid Mechanics: Transport and Diffusion Analyses as Applied in Biomaterials Studies
Abbreviations
3.309.1. Introduction
3.309.2. Transport Phenomena: Diffusive, Convective, and Reactive Transport Mechanisms
3.309.3. Analysis of Transport Models in Porous Media
3.309.4. Conclusion
3.310. Computational Methods Related to Reaction Chemistry
Abbreviations
Acknowledgments
3.310.1. Introduction
3.310.2. Computational Methods
3.310.3. Applications
3.311. Molecular Simulation Methods to Investigate Protein Adsorption Behavior at the Atomic Level
Abbreviations
Acknowledgments
3.311.1. Introduction
3.311.2. Fundamental Aspects of Protein Adsorption Behavior
3.311.3. Molecular Simulation Methods and Their Relevance to Protein Adsorption
3.311.4. Application of All-Atom Modeling Methods to Simulate Protein–Surface Interactions
3.311.5. Current Limitations and Directions for Further Development
3.311.6. Concluding Remarks
3.312. Cell Culture Systems for Studying Biomaterial Interactions with Biological Barriers
Abbreviations
Acknowledgments
3.312.1. Introduction
3.312.2. The Upper Respiratory Tract: Barrier Functions of the Bronchial Epithelium
3.312.3. The Lower Respiratory Tract: Cell Culture Models Mimicking the Biological Barriers of the Distal Lung
3.312.4. In Vitro Studies with Endothelial Cells from the BBB
3.312.5. Conclusion and Future Perspectives
3.313. Histological Analysis
Abbreviations
3.313.1. Introduction
3.313.2. Preservation of Tissue
3.313.3. Undecalcified Versus Decalcified
3.313.4. Embedding Techniques
3.313.5. Sectioning Techniques
3.313.6. Staining Methods
3.313.7. Quantification Methods
3.313.8. Summary and Future Directions
3.314. Materials to Control and Measure Cell Function
Abbreviations
3.314.1. Introduction
3.314.2. Influence of Surface Features on Cell Function
3.314.3. Influence of Surface Features on Bacteria Function
3.314.4. Applications in BioMEMs/Microsystems Fields
3.314.5. Conclusions
3.315. Biological Microelectromechanical Systems (BioMEMS) Devices
Abbreviations
Acknowledgments
3.315.1. Introduction
3.315.2. Cell Adhesions to the Microenvironment
3.315.3. BioMEMS Devices to Measure Traction Forces
3.315.4. BioMEMS Devices to Apply Forces to Cells
3.315.5. Microfluidic Systems
3.315.6. Future Directions
3.316. Immunohistochemistry
Abbreviations
3.316.1. Introduction to Immunohistochemistry
3.316.2. Factors Contributing to Antibody–Antigen Interaction
3.316.3. Visualization of Antibody by Enzymatic or Fluorescent Labeling and The Advantages and Disadvantages
3.316.4. Basic Immunohistochemistry Protocols and Their Advantages and Disadvantages for Biomaterial Science
3.316.5. Detection of Pathobiological Processes and Associated Cellular Events in an Implanted Site
3.316.6. Implant Evaluation Using Immunohistochemical Methods
3.317. Fluorescence Imaging of Cell–Biomaterial Interactions
Abbreviations
Acknowledgments
3.317.1. Introduction
3.317.2. Principles, Instrumentation, and Methodology for Cell Imaging
3.317.3. Probing Cell–Biomaterial Interactions
3.317.4. Looking to the Future
3.318. Molecular Imaging
Abbreviations
3.318.1. Introduction
3.318.2. Nanomaterials
3.318.3. Nanovesicles
3.318.4. Polymeric Assemblies
3.318.5. Future Outlook
3.319. Characterization of Nanoparticles in Biological Environments
Abbreviations
Acknowledgments
3.319.1. Introduction
3.319.2. Interactions of Nanoparticles
3.319.3. Characterization of Nanoparticles in or from Solution
3.319.4. Examples of Nanoparticles in Biological Environments
3.319.5. Conclusions and Outlook
3.320. Nanostructured Polymeric Films for Cell Biology
Abbreviations
3.320.1. Introduction
3.320.2. Controlled Protein Adsorption on BCP and Polymer Blend Surfaces
3.320.3. Protein and Cell Binding via Affinity Interactions Templated by Polymer Surfaces
3.320.4. Coassembly of BCPS with Peptides and Proteins in Thin Films
3.320.5. BCP Surfaces for Biocompatibility and Antifouling Applications
3.320.6. BCP Micelle Surfaces for Controlled Release Applications
3.320.7. Conclusion
3.321. Microarrays in Biomaterials Research
Abbreviations
3.321.1. Introduction
3.321.2. Principle of Microarray
3.321.3. Application of Microarray
3.321.4. Future Prospects of Microarray
3.322. Infrared and Raman Microscopy and Imaging of Biomaterials
Abbreviations
3.322.1. Introduction
3.322.2. Instrumentation, Sampling, and Data Processing
3.322.3. Applications to Biomaterials
3.322.4. Conclusions
3.323. Magnetic Resonance of Bone Microstructure and Chemistry
Abbreviations
3.323.1. Introduction
3.323.2. Fundamentals of Magnetic Resonance
3.323.3. Quantitative MRI of Trabecular and Cortical Bone Microstructure
3.323.4. Bone Water and Porosity
3.323.5. NMR Spectroscopy and MRI of Bone Matrix and Mineral
3.323.6. Summary and Conclusions
3.324. Fluorescent Nanoparticles for Biological Imaging
Acknowledgments
3.324.1. Introduction
3.324.2. Fluorescent Nanoparticles: Synthesis and Surface Modification
3.324.3. Fluorescent Nanoparticles: In Vitro Applications
3.324.4. Fluorescent Nanoparticles: In Vivo Applications
3.324.5. Future Perspective
3.325. Imaging Mineralized Tissues in Vertebrates
Abbreviations
3.325.1. Introduction
3.325.2. Structure of Mineralized Tissues
3.325.3. Heterogeneity of Mineral Content and the Need for Imaging Techniques
3.325.4. Estimates of Total Bone Mineral Content
3.325.5. Imaging of Mineralized Tissues
3.325.6. Property Mapping in Mineralized Tissues
3.325.7. Conclusions
3.326. Imaging and Diagnosis of Biological Markers
Abbreviations
3.326.1. Introduction
3.326.2. Principles of MR and the Implications for Imaging and Diagnosis
3.326.3. Viable Molecules, Nuclei, and Experiments for MR Studies
3.326.4. Case Study: Biological Markers for the Diagnosis of DDD
3.326.5. Case Study: MR for Diagnosing Bone Disease and Treatment
3.326.6. Summary and Future of Imaging and Diagnosis of Biological Markers
3.327. Intracellular Probes
Abbreviations
3.327.1. Introduction
3.327.2. Fluorescence-Based Intracellular Probes
3.327.3. Optical-Label-Free Intracellular Probes
3.327.4. Conclusion
3.328. Biosensors Based on Sol–Gel-Derived Materials
Abbreviations
3.328.1. Introduction
3.328.2. Sol–Gel Bioencapsulation
3.328.3. Applications of Sol–Gel Biosensors
3.328.4. Conclusions and Future Trends
3.329. Hydrogels in Biosensing Applications
3.329.1. Introduction
3.329.2. Physicochemical Sensing Mechanisms
3.329.3. Biochemical Sensing Mechanisms
3.329.4. Summary
3.330. Carbon Nanotube-Based Sensors: Overview
3.330.1. Introduction
3.330.2. CNTs and Related Carbon-Based Nanostructures
3.330.3. Manufacturing Devices from Carbon Nanostructures
3.330.4. Sensing Applications of Carbon Nanostructures
3.330.5. Future Opportunities
3.331. Conjugated Polymers for Biosensor Devices
Abbreviations
3.331.1. Conjugated Polymers (CPs): History and Perspectives
3.331.2. Using CPs for Electrochemical Biosensor Devices
3.331.3. Using CPs for Optical Biosensor Devices
4.401. The Concept of Biocompatibility
Acknowledgment
4.401.1. Introduction
4.401.2. Wound-Healing Responses Underlying Biocompatibility
4.401.3. Characteristic Features of Tissue Around Implants: Emerging Insights
4.401.4. Summary and Considerations for the Future
4.402. Biocompatibility and the Relationship to Standards: Meaning and Scope of Biomaterials Testing
Abbreviations
4.402.1. Introduction
4.402.2. Biocompatibility
4.402.3. Materials for Medical Devices
4.402.4. In Vitro Tests for Biocompatibility
4.402.5. In Vivo Tests for Biocompatibility
4.402.6. Inflammation, Wound Healing, and the Foreign-Body Response
4.402.7. Hemocompatibility
4.402.8. Immune Responses
4.402.9. Summary and Conclusion
4.403. The Innate Response to Biomaterials
Abbreviations
4.403.1. Introduction
4.403.2. Biomaterial Changes
4.403.3. Inflammation and Biomaterials
4.403.4. Evasion Innate Immune System Activation
4.403.5. Enhancement of Innate Immune System Activation
4.403.6. Concluding Remarks
4.404. Adaptive Immune Responses to Biomaterials
4.404.1. Introduction
4.404.2. Recognition of Biomaterials by the Adaptive Immune System
4.404.3. Consequences of the Adaptive Response
4.404.4. Approaches to Engineer Adaptive Responses with Biomaterials
4.404.5. Conclusions
4.405. Leukocyte–Biomaterial Interaction In Vitro
Abbreviations
4.405.1. Introduction
4.405.2. Characterizing the Inflammatory Response
4.406. Protein Interactions with Biomaterials
4.406.1. Introduction
4.406.2. Surface Wettability, Topography, and Protein Adsorption
4.406.3. Surface-Activated Coagulation
4.406.4. Immune Complement at Biomaterials
4.406.5. Consequences of Protein Adsorption
4.406.6. Summary and Future Directions
4.407. Bacterial Adhesion and Biomaterial Surfaces
Abbreviations
4.407.1. Introduction
4.407.2. Laboratory Methods for the Study of Bacterial Adhesion
4.407.3
- Edition: 1
- Published: August 24, 2011
- Imprint: Elsevier Science
- Language: English
PD
Paul Ducheyne
PD
Paul Ducheyne
Paul Ducheyne is Professor of Bioengineering and Professor of Orthopaedic Surgery Research at the University of Pennsylvania, Philadelphia, USA. He is the Director of its Center for Bioactive Materials and Tissue Engineering. He also is Special Guest Professor at the University of Leuven, Belgium.
Paul Ducheyne has Materials Science and Engineering degrees from the K.U. Leuven. Belgium (M.Sc.: 1972; Ph.D.: 1976). With fellowships from the National Institutes of Health (International Postdoctoral Fellowship) and the Belgian American Educational Foundation (Honorary Fellowship), he performed postdoctoral research at the University of Florida.
Paul Ducheyne has organized a number of symposia and meetings, such as the Fourth European Conference on Biomaterials (1983), the Engineering Foundation Conference on Bioceramics (1986) which led to the New York Academy of Sciences publication: "Bioceramics, material characteristics versus in vivo behavior", and the Sixth International Symposium on Ceramics in Medicine (1993). He has lectured around the world and serves or has served on the editorial board of more than ten scientific journals in the biomaterials, bioceramics, bioengineering, tissue engineering, orthopaedics and dental fields. He has been a member of the editorial board, and then an associate editor of Biomaterials, the leading biomaterials journal, since its inception in the late seventies. He has authored more than 300 papers and chapters in a variety of international journals and books, and he has edited 10 books. He has also been granted more than 40 US patents with international counterparts. His papers have been cited about 7000 times; his ten most visible papers have been cited more than 2000 times.
Paul Ducheyne started his career in Europe. While at the K.U. Leuven, Belgium (1977 - 1983), he was one of the co-founders of the Post-Graduate Curriculum in Bioengineering. This program is now a full M.Sc. program in the School of Engineering and Applied Sciences. In those initial years, he was also chairman-founder of the chapter on Biomedical Engineering of the Belgian Engineering Society (Flemish section) and director of Meditek, the Flemish Government body created to promote Academia to Industry Technology Transfer in the area of Biomedical Engineering.
Paul Ducheyne founded Gentis, Inc., which focuses on breakthrough concepts for spinal disorders. Previously, he founded Orthovita (NASDAQ: VITA) in 1992 and served as Chairman of its Board of Directors until 1999. Orthovita focuses on bioceramic implant materials for orthopaedics.
Paul Ducheyne has been secretary of the European Society for Biomaterials, is Past President of the Society for Biomaterials (USA) and Past President of the International Society for Ceramics in Medicine. He has been recognized as a fellow of the American Association for the Advancement of Science (AAAS), fellow of the American Institute of Medical and Biological Engineering (AIMBE), and fellow of the International Association of Biomaterials Societies. He was the first Nanyang Visiting Professor at the Nanyang Institute of Technology, Singapore and he has received the C. William Hall Award from the Society for Biomaterials.
Many of Paul Ducheyne's trainees have become leaders of the next generation. Among his trainees are professors at the University of California at Berkeley, the University of Michigan, Columbia University, Georgia Institute of Technology, the K.U. Leuven (Belgium), etc... Among the six U.S. Associate Editors of the Journal for Biomedical Materials Research (the Journal of the Society for Biomaterials), three were his PhD students.
KH
Kevin Healy
DH
Dietmar W. Hutmacher
DG
David W. Grainger
David W. Grainger is the George S. and Dolores Dore´ Eccles Presidential Endowed Chair in Pharmaceutics and Pharmaceutical Chemistry, past Chair of the Department of Pharmaceutics and Pharmaceutical Chemistry, and Chair and Professor of Bioengineering at the University of Utah, USA.
Grainger received his Ph.D. in Pharmaceutical Chemistry from the University of Utah in 1987. With an Alexander von Humboldt Fellowship, he undertook postdoctoral research in biomembrane mimicry and assembly under Prof. Helmut Ringsdorf, University of Mainz, Germany.
Grainger’s research focuses on improving implanted medical device performance, drug delivery of new therapeutic proteins, nucleic acids and live vaccines, nanomaterials interactions with human tissues, low-infection biomaterials, and innovating diagnostic devices based on DNA and protein biomarker capture. He also has expertise in perfluorinated biomaterials and applications of surface analytical methods to biomedical interfaces, including surface contamination, micropatterns, and nanomaterials.
Grainger has published over 190 research papers at the interface of materials innovation in medicine and biotechnology, and novel surface chemistry. He has organized many international scientific symposia and chaired the Gordon Research Conference in Biomaterials and Tissue Engineering. He frequently lectures worldwide, including delivering many named, keynote, and plenary presentations.
Grainger serves on the editorial boards of four major journals in the biomedical materials field. He is currently a Council member at the National Institutes of Health, and has served on many national and international review panels, including the NIH’s Surgery and Bioengineering and Emerging Bioanalytical and Imaging Technologies Scientific Review Groups. He remains active on academic scientific advisory boards for diverse academic programs in the United States, Asia, and Europe, including major research centers at the Universities of Wisconsin-Madison and University of Washington, the AO Foundation and EMPA, Switzerland, the Charité, Germany, several other competence centers in Europe.. Grainger also sits on the scientific advisory boards for four biomedical companies and actively consults internationally with industries in applications of materials in biotechnologies and medicine.
His scientific and technical accomplishments are widely recognized, both at his institution and worldwide. Among several citations, Grainger is fellow of the American Association for the Advancement of Science (AAAS), the American Institute of Medical and Biological Engineering (AIMBE), and the International Union of the Societies of Biomaterials Science and Engineering. He has also been honored with the 2007 Clemson Award for Basic Research, Society for Biomaterials, and the 2005 American Pharmaceutical Research and Manufacturer’s Associa- tion’s award for ‘Excellence in Pharmaceutics’.
CK
C. James Kirkpatrick
C. James Kirkpatrick is Emeritus Professor of Pathology at the Johannes Gutenberg University of Mainz, Germany, having directed the Institute of Pathology from 1993-2015. Currently he is Senior Professor in the Cranio-Maxillofacial Surgery Clinic at the Goethe University of Frankfurt & Visiting Professor of Biomaterials & Regenerative Medicine at the University of Gothenburg, Sweden. He is also Honorary Professor at the Peking Union Medical College, Beijing and the Sichuan University, Chengdu, China.
Kirkpatrick is a graduate of the Queen’s University of Belfast and holds a triple doctorate in science and medicine (PhD: 1977; MD: 1982; DSc: 1992). Previous appointments were in pathology at the University of Ulm, where he did postdoctoral research in experimental pathology, Manchester University (Lecturer in Histopathology) and the RWTH Aachen (Professor of Pathology & Electron Microscopy).
On moving to Aachen in 1987, he established a cell culture laboratory which began using modern methods of cell and molecular biology to study how human cells react to biomaterials. Since then, his principal research interests continue to be in the field of biomaterials in tissue engineering and regenerative medicine, with special focus on the development of human cell culture techniques, including novel 3D coculture methodology for biomaterials and the application of modern molecular pathology techniques to the study of biofunctionality of biomaterials, including nanomaterials.
Kirkpatrick is author/coauthor of more than 500 publications in peer-reviewed journals and has given more than 500 invited presentations to scientific meetings worldwide. He has an H-index of 58 (Web of Science) and 68 (Google Scholar) and has been cited more than 17.000 times.
He is a former president of both the German Society for Biomaterials (2001–2005) and the European Society for Biomaterials (2002–2007) and served on the ESB council from 1995-2013. He was also a member of the Council of the European Chapter of the Tissue Engineering & Regenerative Medicine International Society (TERMIS-EU; 2006-2008; 2010-2012).
Kirkpatrick was a long-standing member of the editorial board of the premier journal Biomaterials (1996-2014) and also associate editor (2002-2014). He has also served as associate editor of the leading Journal of Pathology (2001–2006). In total, he serves or has served as an editorial board member of 18 international journals in pathology, biomaterials, and tissue engineering.
Kirkpatrick was the Scientific Programme Committee Chair for the 8th World Biomaterials Congress in Amsterdam in 2008.
Kirkpatrick is a member of the Scientific Advisory Board of a number of research institutes, centres of excellence and companies in biomaterials and regenerative medicine in Europe, as well as the Medical Technology Committee, Federal Ministry of Education & Research in Germany (BMBF) (2005-2008) and the German Federal Institute for Drugs & Medical Devices (BfArM)(since 2007).
Kirkpatrick has been recognized for his contributions. He is a Fellow of the Royal College of Pathologists, London and a Fellow of Biomaterials Science & Engineering (FBSE) of the IUS-BSE (International Union of Societies for Biomaterials Science & Engineering). He received the Research Prize of the State of Rhineland-Palatinate for Research on Replacement and Alternative Methods for Animal Research. He was the recipient of the George Winter Award from the European Society for Biomaterials (2008), and in 2010, he received, as first medical graduate, the Chapman Medal from the Institute of Materials, Minerals & Mining in London for “distinguished research in the field of biomedical materials”. In 2014 Kirkpatrick received the TERMIS-EU Career Achievement Award.